For centuries, humans have sought to understand—and potentially slow, halt or reverse—the aging process. Recent advances in molecular and cellular biology have begun to illuminate the core hallmarks and drivers of aging, providing a foundational framework for new therapeutic approaches. While some of these therapies have not fulfilled their initial promise, others have achieved remarkable progress, and ongoing clinical trials may pave the way for a new class of longevity drugs. Still, despite growing optimism, the journey toward regulatory approval and widespread adoption of these treatments presents a unique set of challenges that must be navigated carefully.
February 2025
As a science-driven venture fund, we often have the pleasure of meeting and working with dedicated researchers who tirelessly strive to unlock new insights within human health, and no other field is as fascinating, complex and provocative as the field of aging. This emerging therapeutic space will affect all humans and is woven into the fabric of the human experience.
In this white paper, our team takes a deep dive into aging, longevity research and drug development. We delve into our evolving understanding of the mechanisms behind aging, examine the challenges that hinder the development of effective therapeutics and highlight the promising opportunities emerging in this rapidly advancing field, including the cutting-edge research being performed at Altos Labs, one of the Luma Group’s portfolio companies.
What is aging: unraveling a whole-body disease
We all have or will experience symptoms of aging, from stiffening joints to moments of forgetfulness, but what is the biology driving this seemingly inevitable process? Humans have studied aging for thousands of years but have just begun to understand the underlying causes of the aging process. The most significant advances have come with the help of modern medicine and science and have begun to shed light on core drivers in the aging process. Although we have begun to make headway in understanding aging, there is still a vast amount that we don’t understand but are hopeful of uncovering within our lifetimes.
Thanks to groundbreaking advances in molecular biology techniques, our understanding of aging has moved from speculation to a science rooted in cellular mechanisms. These technologies have allowed researchers to dissect the intricate molecular pathways that drive aging, unlocking insights into the cellular damage, DNA repair deficits and protein misfolding that contribute to the process. What was once seen as a vague, inevitable decline is now recognized as a complex, multi-system condition with identifiable triggers. As we continue to decode these molecular signals, we edge closer to the possibility of interventions that could delay or even reverse the effects of aging.
What do we know: the molecular hallmarks of aging
Aging is a complex, multifaceted process that affects every cell and tissue in the human body. Over the past few decades, significant advances in molecular biology have allowed researchers to identify and characterize the core mechanisms driving this inevitable decline.
These mechanisms, known as the molecular hallmarks of aging, provide critical insights into how our bodies change over time. By understanding these hallmarks—ranging from genomic instability to mitochondrial dysfunction—scientists are uncovering potential targets for interventions aimed at slowing, or even reversing, the aging process. In this section, we explore the key molecular drivers of aging and how they impact human health and longevity and what promise they hold for drug development.
Graphic 1: The molecular hallmarks of aging.
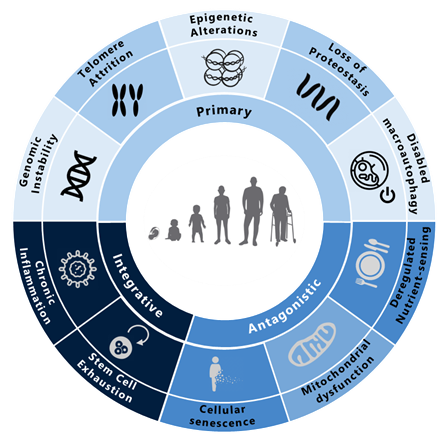
Source: https://www.sciencedirect.com/science/article/pii/S0092867422013770.
Genomic Instability
Aging is closely linked to increased genomic instability, characterized by the accumulation of mutations, chromosomal abnormalities and DNA damage. These genetic insults arise from multiple sources, including replication errors, environmental factors, such as stress, radiation and toxins, as well as endogenous factors like reactive oxygen species (ROS) produced during normal cellular metabolism. Over time, the continual onslaught of genomic damage compromises the integrity of both nuclear and mitochondrial DNA, driving cellular malfunction, senescence and an elevated risk of cancer.
Graphic 2: Drivers of genomic instability and their effect on aging.
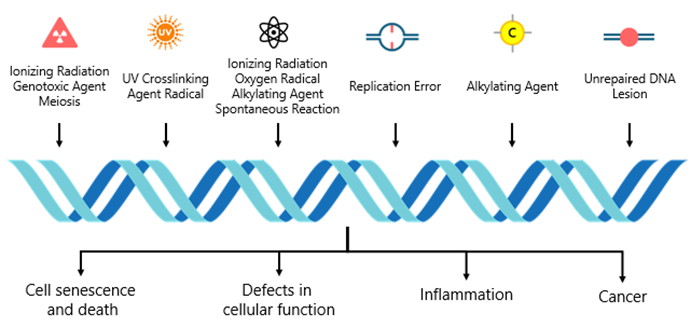
Source: https://www.sciencedirect.com/science/article/abs/pii/B9780128237618000203?via%3Dihub
Data from animal models underscore the profound impact of genomic integrity on longevity. For instance, transgenic mice overexpressing BubR1—a mitotic checkpoint regulator—demonstrated increased lifespans and a reduced incidence of age-related diseases.1 Similarly, enhancing DNA repair and genomic stability through genes like SIRT6 has been shown to improve lifespan in mice by mitigating the accumulation of DNA damage.2 Conversely, increasing genomic mutations in mice by altering DNA proofreading proteins leads to phenotypes resembling accelerated aging, including alopecia, kyphosis, osteoporosis, anemia, reduced fertility and cardiac enlargement. Related insights from human cells and progeroid syndromes (e.g., Werner’s syndrome) further confirm that defects in DNA repair mechanisms hasten aging.3
Despite these findings, pinpointing the root causes and precise mechanisms linking genomic instability to aging remains exceptionally complex. Many of the underlying pathways are shared by both healthy and aged cells, making it difficult to identify interventions that selectively improve genomic integrity without adversely affecting normal functions. Determining the optimal timing, method and location for DNA repair interventions is another formidable challenge; correcting one issue may inadvertently trigger new ones. Although numerous studies have reinforced the connection between genetic instability and age-related decline, they often fail to yield clear therapeutic targets or actionable pathways. As a result, developing safe and targeted strategies to address genomic instability—without introducing additional risks—remains a major hurdle in translating these insights into effective anti-aging therapies.
Telomere Attrition
Telomeres, repetitive DNA sequences that cap the ends of chromosomes, progressively shorten with each cell division due to the “end-replication problem.” Once telomeres reach a critically short length, cells are driven into senescence or apoptosis—an important safeguard against unchecked cell proliferation and cancer. Yet, this protective mechanism also contributes to tissue dysfunction as we age, linking telomere shortening to various age-related diseases such as cardiovascular disease, osteoporosis and immunosenescence.
While it is clear that telomeres play a role in aging, the precise nature of their influence remains uncertain. On one hand, shortening telomeres can push cells toward senescence, potentially driving age-related pathologies. On the other hand studies in animal models show that restoring telomerase activity can prevent telomere shortening and even reverse certain signs of aging, effectively extending lifespan in telomerase-deficient mice.4 Research on telomere length in human populations similarly links short telomeres with an increased risk of age-related diseases such as pulmonary fibrosis.5 Additionally, studies involving “hyperlong” telomeres in mice have revealed improved metabolic health and longer lifespans.6 Together, these findings underscore telomeres as regulators of the aging process.
Graphic 3: Cellular cascade of telomere shorting.
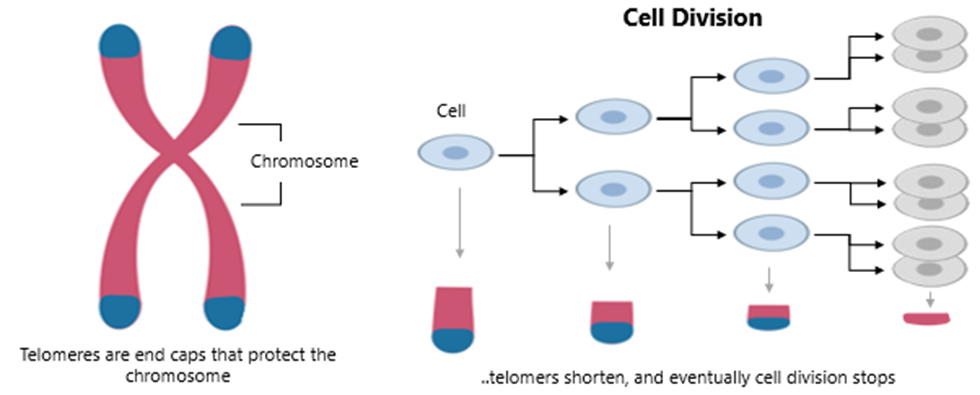
Source: https://sciencellonline.com/blog/aging-and-telomere-length-quantification-by-qpcr/
However, the relationship between telomere length and aging is far from straightforward. Some evidence suggests that individuals with relatively short telomeres may still live longer than those with longer telomeres, indicating that telomere length alone may not reliably predict healthspan (the healthy part of a lifespan) or lifespan (the total span of life).7 Rather than functioning as a simple “molecular clock,” telomeres likely interact with diverse cellular pathways, contributing to aging through multiple and sometimes contradictory mechanisms.
Complicating matters further, the dual role of telomeres in both cancer prevention and cancer promotion creates significant challenges for translating these insights into clinical therapies. Telomerase activation might slow or even reverse certain aging phenotypes, yet it could also enable cells to proliferate beyond their natural limits, increasing cancer risk. In essence, telomeres present a double-edged sword: although they offer potential avenues for intervention, such approaches must navigate the intricate balance between mitigating age-related decline and avoiding oncogenic transformation. Although the basic science surrounding telomeres is intriguing, these complexities highlight the need for a deeper biological understanding before safe and effective therapeutic strategies can be developed.
Epigenetic Alterations
Epigenetic changes—including DNA methylation, histone modifications and chromatin remodeling—adjust gene expression without altering the underlying DNA sequence. As we age, these epigenetic landscapes become increasingly disrupted, influencing cell identity, function and overall health. Such alterations have been implicated in a variety of age-related diseases, including cancer, cardiovascular conditions and neurodegenerative disorders. Using “epigenetic clocks” based on DNA methylation patterns can predict biological age, and administration of α-ketoglutarate has been shown to reduce the epigenetic age of cells by eight years.8 In animal models, inactivating histone acetyltransferase Kat7 delays cellular aging and extends lifespan, while overexpressing SIRT1 improves genomic stability and promotes healthier, longer life.9,10
Graphic 4: Age-related alterations and underlying mechanisms.
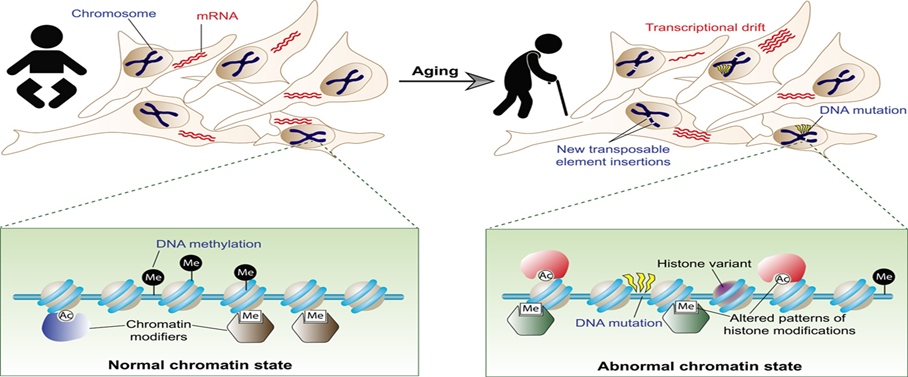
Source: https://www.science.org/doi/10.1126/sciadv.1600584
Despite these promising findings, the field of epigenetics in aging is still in its early stages. Establishing whether epigenetic modifications are genuinely causal—and not merely correlational—requires larger and more comprehensive datasets. Complicating this effort, epigenetic changes are influenced by a combination of genetic and environmental factors, making it challenging to isolate individual contributors. Although epigenetic markers currently serve as intriguing potential biomarkers for aging, significant maturation and validation of this research are needed before these discoveries can be translated into safe, effective therapeutic interventions.
Loss of Proteostasis
Proteostasis involves maintaining the delicate balance of protein synthesis, folding and degradation required for proper cellular function. As organisms age, this balance deteriorates, allowing misfolded or damaged proteins to accumulate and form toxic aggregates, such as the amyloid plaques seen in Alzheimer’s disease. The breakdown of proteostasis has also been implicated in various age-related conditions, including cataracts and sarcopenia.
Graphic 5: Protein aggregation driven mechanisms of aging
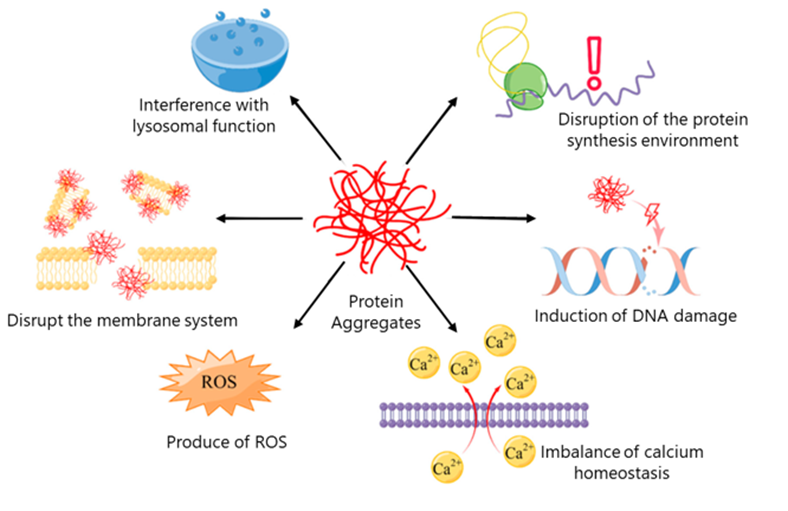
Source: https://www.mdpi.com/1422-0067/24/10/8593
Several approved and clinical-stage therapies aim to modify proteostasis machinery directly. For instance, the proteasome inhibitor bortezomib, originally approved for oncology, effectively treats certain lymphomas and multiple myeloma by halting protein degradation and inducing cancer cell death. More recently, a growing focus has turned toward enhancing, rather than inhibiting, proteasome activity. By boosting the cell’s capacity to clear harmful proteins, these activator therapies may offer new strategies for combatting age-associated diseases. Real-world data reinforce the therapeutic potential of improving proteostasis. Overexpressing LAMP2A, a regulator of chaperone-mediated autophagy, improved proteostasis and extended lifespan in mice.11 Similarly, administration of recombinant HSP70 enhanced proteasome activity, reduced brain lipofuscin—a hallmark of aging—and increased lifespan in mouse models.12 These findings suggest that therapies targeting protein quality control mechanisms could significantly delay the onset and progression of age-related diseases.
However, there are still numerous challenges in developing proteostasis therapeutics for aging. While current hypotheses link declining proteasome activity to aging and disease states, it remains uncertain whether restoring proteostasis in humans will yield the same benefits observed in animal models. Developing effective proteasome activators has proven more complex than creating inhibitors, given the intricate biology of these pathways and the difficulty of achieving selective targeting. Nonetheless, several proteasome activators are moving through clinical development, and their outcomes will help determine whether proteostasis-enhancing interventions can overcome these therapeutic hurdles—and ultimately improve health span and longevity.
Disabled Autophagy
Autophagy, a cellular quality control mechanism, degrades and recycles damaged organelles and other cellular components to maintain overall cellular health. By preventing the accumulation of waste and facilitating the turnover of older or malfunctioning organelles to preserve cellular function. However, as we age, this process becomes less efficient, contributing to the onset and progression of age-related conditions, including neurodegenerative diseases, cancer and metabolic disorders.
Graphic 6: the role of autophagy in cellular health.
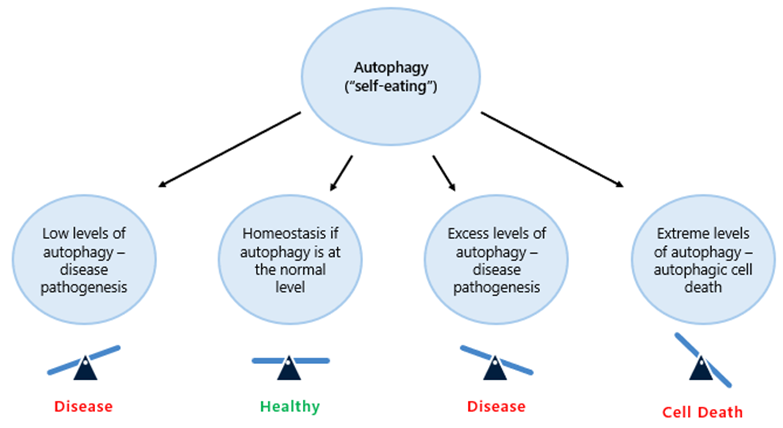
Source: https://www.preprints.org/manuscript/202303.0500/v1.
In mice, overexpressing the autophagy-related gene Atg5 extends lifespan and improves metabolic health.13 Similarly, spermidine, a naturally occurring compound that induces autophagy, has been shown to lengthen lifespan and mitigate age-related cardiac dysfunction.14 Further research has demonstrated that pharmacological agents like salicylates, which promote autophagy by inhibiting EP300, can enhance autophagic activity and increase longevity in mice.15
Despite these promising findings, the therapeutic potential of enhancing or restoring autophagy remains challenging to harness. Much like the complexities faced in targeting proteostasis, interventions aimed at modulating autophagy must navigate intricate biological networks and ensure selectivity and safety. Although GWAS data from diseases like ALS and Parkinson’s support the rationale for restoring proper autophagy levels, the difficulty in identifying suitable intervention points—and the need to avoid unintended consequences—continues to pose significant hurdles. As research advances, overcoming these challenges will be critical for translating the science of autophagy into effective therapies against age-related diseases.
Mitochondrial Dysfunction
Mitochondria are central to cellular function, not only as the primary generators of ATP but also as regulators of metabolism, reactive oxygen species (ROS) production and cell death. As organisms age, mitochondrial function declines, resulting in diminished energy production and increased ROS, both of which contribute to cellular damage and aging. This decline in mitochondrial health is associated with a broad spectrum of age-related pathologies, including neurodegenerative, cardiovascular and metabolic diseases. Interventions have emerged that target mitochondrial function to extend healthspan. For instance, supplementation with NAD+ precursors such as nicotinamide mononucleotide (NMN) has improved mitochondrial function, boosted energy levels and extended lifespan in mice.16 Similarly, the drug elamipretide, developed to protect mitochondrial membranes, has shown improvements in heart and muscle function in both aged mice and humans.17 These examples suggest that strategies aimed at sustaining or restoring mitochondrial health could delay aging and mitigate age-related diseases.
Graphic 7: The mechanisms of action of mitochondrial dysfunction in aging and disease.
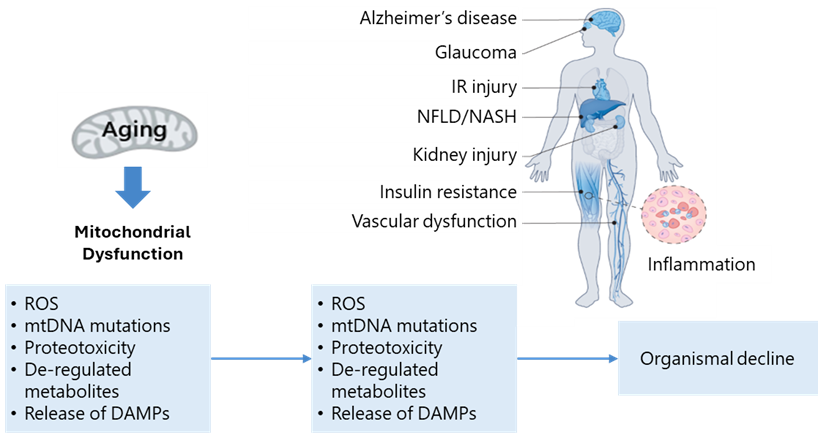
Source: https://www.nature.com/articles/s43587-022-00191-2#Sec2
However, effective therapies must also consider the maintenance and removal of damaged mitochondria through mitophagy—an autophagy subtype dedicated to mitochondrial quality control. Mitophagy-targeted interventions face challenges akin to those encountered in general autophagy-based therapies, necessitating enhanced or activated protein degradation pathways capable of efficiently clearing dysfunctional organelles. Mitochondria contain their DNA, thus opening additional avenues for intervention through the modulation of mitochondrial polymerases and other enzymes. While numerous drug candidates focusing on mitochondrial health have progressed into clinical trials, none have yet secured FDA approval. Nevertheless, the results of these ongoing trials—whether successful or not—will likely illuminate the most promising mechanisms of action for improving mitophagy and mitochondrial function.18
Deregulated Nutrient-Sensing
Nutrient-sensing pathways—including insulin/IGF-1, mTOR and AMPK—are central regulators of metabolism and growth, constantly adjusting cellular processes in response to nutrient availability. During aging, however, deregulation of these pathways often leads to excessive anabolism and reduced autophagy, accelerating the aging process and increasing susceptibility to metabolic diseases like diabetes. Interventions that reduce nutrient signaling, such as caloric restriction or pharmacological inhibitors of nutrient-sensing pathways, have consistently extended lifespan across multiple species. For example, in human studies, two years of caloric restriction improved markers of inflammation and enhanced immune function, underscoring its potential to slow aging.19 In mice, pharmacological inhibition of mTOR with rapamycin has been shown to prolong lifespan and improve age-related outcomes, while metformin—a common diabetes medication that modulates nutrient-sensing pathways—is currently under investigation for its anti-aging potential in human trials.20,21
Collectively, these findings suggest that interventions targeting nutrient-sensing pathways hold promise for extending lifespan and mitigating age-related diseases. The prevailing hypothesis is that these strategies induce beneficial stress responses, such as enhanced protein and mitochondrial turnover, thereby promoting cellular resilience and longevity. Still, determining the true magnitude of these benefits presents challenges, as it entails altering diets or administering drugs in highly heterogeneous aging populations with diverse health conditions over a very long time. Large-scale, extended-duration clinical trials are necessary to gain a more definitive understanding of their long-term effects. Some of these investigations are already in progress, but it may be years before their outcomes are known. Only then will we have a clearer picture of whether interventions like caloric restriction or low-dose metformin can reliably increase lifespan or significantly improve outcomes in age-related conditions.
Cellular Senescence
Cellular senescence occurs when cells cease to divide but remain metabolically active, secreting inflammatory and damaging factors that negatively affect surrounding cells and tissues. This accumulation of senescent cells is strongly implicated in age-related diseases, including osteoarthritis, atherosclerosis and neurodegeneration. Removing senescent cells, therefore, represents a promising therapeutic approach to mitigating the adverse effects of aging and age-related pathologies. Multiple lines of evidence indicate that these cells build up as we get older, and their targeted elimination can improve the overall health and function of tissues and organs. For example, senolytic drugs—therapies designed to selectively clear senescent cells—have shown success in animal models; the combination of dasatinib and quercetin, for instance, reduced the number of senescent cells in aged mice, improving markers of aging.22 Early human trials of senolytics have also reported encouraging results, demonstrating reduced senescence markers and improved health outcomes in age-related conditions.23
Graphic 8: Mechanism of action for senoltyics in aging.
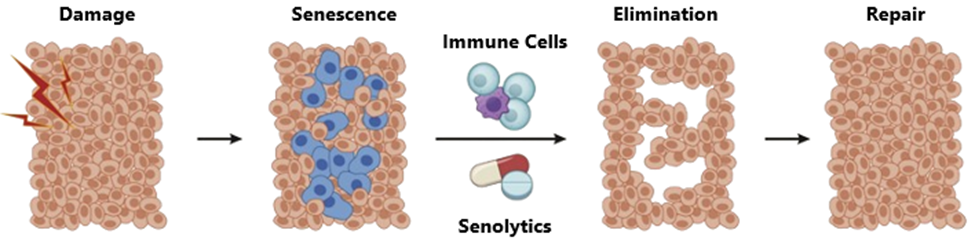
Source: https://computationalaginglab.github.io/computational_aging_course/bio/intro_aging_biology.html
Although the field of senolytics is still in its infancy, it holds significant promise. Like oncology, it benefits from the presence of a distinct pathological cell type as a target. Still, a major challenge lies in pinpointing the precise therapeutic nodes or targets that can effectively and selectively eliminate senescent cells, allowing tissues and organs to renew themselves with healthy, functional counterparts. Our current understanding of senescent cell biology remains limited, and the identification of specific, compelling targets is an ongoing endeavor. As research advances, filling these gaps in knowledge may pave the way for the development of safe and effective senolytic therapies.
Stem Cell Differentiation and Reprograming
Stem cells possess a remarkable capacity for self-renewal and differentiation into virtually any cell type, with each organ containing a specialized stem cell niche that generates new cells to replace those that are damaged or aged. This regenerative capacity is central to tissue repair and maintenance; however, it diminishes over time. As people grow older, stem cell activity declines, resulting in compromised tissue integrity and an elevated risk of diseases such as cancer and cardiovascular disorders. This phenomenon, termed stem cell exhaustion, arises from both intrinsic factors (e.g., DNA damage) and extrinsic factors (e.g., altered stem cell niches and systemic inflammation). Despite these challenges, stem cells remain highly promising therapeutic tools because they offer the potential to restore damaged or aging tissues using the body’s own healthy cells. Over the past two decades, researchers have made substantial progress in deriving stem cells from adult cells and guiding them to form a wide range of specialized cell types, thereby laying the groundwork for new treatments that may mitigate many aspects of age-related decline.
Graphic 9: Yamanaka factor stem cell reprograming and terminal differentiation.
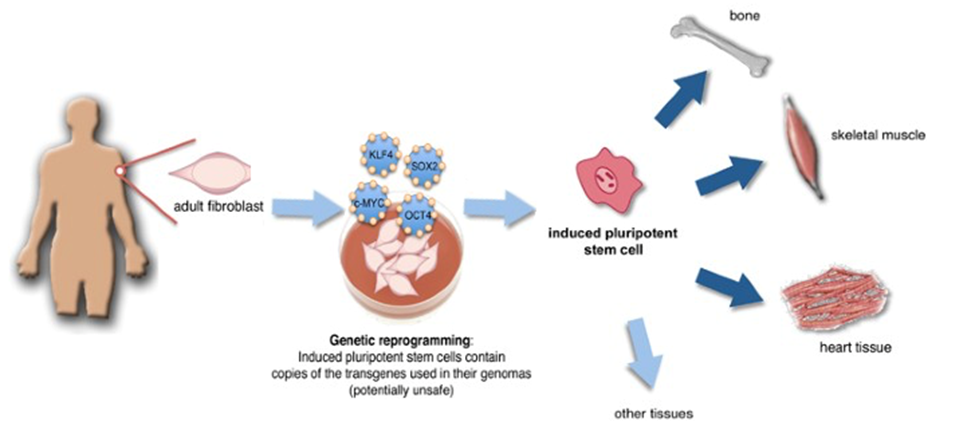
Source: adapted from https://www.nature.com/articles/cdd201014.
A groundbreaking leap in this field came in 2006, when Dr. Shinya Yamanaka identified four key transcription factors—Oct4, Sox2, Klf4 and c-Myc—that could reprogram ordinary adult cells into induced pluripotent stem cells (iPSCs), resetting them to a stem cell-like state.24 This discovery revolutionized the scientific landscape by offering an ethically sound and widely accessible alternative to embryonic stem cells, upending the long-standing belief in fixed cellular destinies. With iPSCs, scientists now have unprecedented opportunities to regenerate damaged tissues or organs from a patient’s own cells, sharply reducing immune rejection risks. This advance also underpins personalized medicine strategies aimed at treating conditions ranging from neurodegenerative diseases to heart disorders. Beyond direct therapeutic applications, iPSCs enable researchers to more accurately model human development and disease, as well as to test drugs on human-like tissues. Yamanaka’s work, which earned him a Nobel Prize, remains at the forefront of regenerative medicine. The impact of his discovery is evident not only in academic research but also in its translation toward clinical applications, exemplified by its central role in the strategy of one of Luma Group’s portfolio companies, Altos Lab, which aims to harness the power of cell reprogramming for therapeutic purposes.
Stem cell technologies thus hold immense potential for addressing many core challenges of aging by replacing or reprogramming pathological cells across a spectrum of age-related diseases. However, several critical obstacles remain. Scaling up cell production and ensuring efficient, precise delivery methods pose significant hurdles for cell replacement therapies (as discussed in the opportunity section). In vivo reprogramming may streamline this process by circumventing the need for large-scale cell manufacturing and intricate delivery protocols. Yet, this approach faces its own difficulties, especially the safe and reliable delivery of multiple reprogramming factors. Notably, this challenge is not unique to longevity research; the gene therapy sector is actively developing solutions that can be used for longevity. As such, continued investment in understanding stem cell biology, refining cell production and improving delivery mechanisms will be essential to fully realize the promise of stem cell–based and reprogramming-based interventions for aging.
Longevity Therapeutics: Challenges from Benchtop to Bedside
Developing therapeutics and conducting clinical trials for longevity presents unique challenges due to the complexity of aging as a biological process. As highlighted in the previous section, aging is influenced by numerous interconnected factors, including genetics, lifestyle and environmental influences, making it difficult to isolate specific targets for intervention. Additionally, the long timescales required to observe meaningful outcomes in human aging, along with the diversity in how individuals age, further complicate the design and execution of clinical trials. Even with these complexities, driven researchers and biotechs have been working on developing longevity therapeutics.
Running clinical trials for longevity therapies poses unique challenges (outlined below) that set them apart from traditional trials. Designing a study to demonstrate a direct extension of lifespan is inherently difficult, so many trials instead focus on specific age-related diseases as more measurable endpoints. Despite these obstacles, several clinical trials targeting age-associated conditions and interventions that may influence lifespan have been conducted, as summarized in the table below.
Length and Complexity of Trials
One of the primary challenges of longevity trials is their extended duration. Since these therapies aim to delay or prevent age-related decline, it may take years, if not decades, to see meaningful results. This long timeframe complicates the trial design, making it difficult to maintain patient adherence and gather consistent data over extended periods. Additionally, many aging-related processes unfold over a person’s lifetime, requiring researchers to use surrogate biomarkers rather than waiting for hard outcomes like mortality or disease onset.
Recruitment and Patient Population
Recruiting participants for longevity trials can be challenging due to the need for a healthy population rather than individuals already diagnosed with a specific condition. Identifying the right demographic—those who are at risk of age-related diseases but have not yet developed them—requires extensive screening and a clear understanding of the aging process. Moreover, convincing healthy individuals to participate in long-term trials, especially with the uncertainty of benefits, can be difficult.
Regulatory Considerations
Longevity research exists at the intersection of prevention and treatment, often challenging traditional regulatory frameworks. Aging is not classified as a disease, which complicates the approval process for therapies aimed at treating it. Regulatory agencies like the FDA do not yet have clear guidelines for evaluating interventions designed to slow aging. This creates significant uncertainty for companies attempting to bring longevity therapies to market.
Biomarkers and Measuring Success
Unlike traditional clinical trials, which measure success based on clear clinical outcomes like symptom improvement or disease remission, longevity trials require novel endpoints. Researchers must rely on biomarkers of aging—such as telomere length, epigenetic changes, or cellular senescence markers—to measure the efficacy of a therapy. Furthermore, these biomarkers also require dedicated development and regulatory approval, often involving extensive and time-consuming human testing. These biomarkers are still under development and debate, leading to potential variability in results and slower progress in validating new treatments.
Logistical Challenges
The logistics of running a longevity trial introduce another layer of complexity. Maintaining patient engagement over the span of years is difficult, especially when participants are often healthy at the start of the study and may not experience immediate benefits. Patient retention is crucial, yet drop-off rates can be high due to the lengthy commitment required. Furthermore, managing data collection across diverse populations over time presents significant challenges, particularly when studies are conducted across multiple geographic locations. Ensuring consistent quality control, adhering to standardized protocols, and synchronizing data from various sites are all critical to the integrity of these studies but are operationally demanding.
Examples of Real World Clinical Trials
Table 1: Overview of past age-related clinical trials.
Objective | Status | Why it’s Significant | |
TAME (Targeting Aging with Metformin) Trial | Tests whether metformin can slow aging and delay age-related diseases such as heart disease, cancer and cognitive decline. | Ongoing, with efforts to get FDA approval to classify aging as an indication. | This trial aims to create a new regulatory path for anti-aging therapies by targeting aging rather than specific diseases. |
UNITY Biotechnology Senolytics Trials | Evaluating the effectiveness of the mechanism of action for senolytics | UBX0101 was tested in patients with osteoarthritis, however it did not show significant improvements. | This was a prominent trial testing the senolytic hypothesis |
CALERIE (Comprehensive Assessment of Long-term Effects of Reducing Intake of Energy) | A long-term trial investigating the effects of caloric restriction on markers of aging, metabolic health and lifespan in humans. | The trial showed calorie restriction caused a significant reduction in all measured conventional cardiometabolic risk factors | First long-term study of caloric restriction in non-obese humans and has contributed valuable data on dietary interventions influence aging. |
ResTORbio RTB101 Trial | Evaluate RTB101 (an mTOR modulator) effects to enhance immune function in the elderly and reduce the incidence of respiratory infections. | Despite promising early results, the Phase 3 trial failed to meet its primary endpoint and development was discontinued. | The mTOR pathway is one of the most well-studied mechanisms of aging and the study aimed to translate animal research into human longevity therapies. |
GRF6019 and GRF6021 (Plasma Dilution Therapy) | These studies aim to evaluate the effectiveness of young plasma treatments on the effects of cognitive decline and motor activity in Alzheimer’s and Parkinson’s disease. | Both trials showed good tolerability but mixed efficacy. The Alzheimer’s trial revealed no significant improvement, while the Parkinson’s trial hinted at early efficacy. Larger studies are needed to determine overall effectiveness. | This trial taps into the intriguing concept of “parabiosis” and seeks to understand whether modifying blood plasma can rejuvenate aging tissues. |
Source: https://www.afar.org/tame-trial, https://pmc.ncbi.nlm.nih.gov/articles/PMC10168460/, https://pmc.ncbi.nlm.nih.gov/articles/PMC3525758/, https://www.sciencedirect.com/science/article/pii/S246850112030002X, https://pubmed.ncbi.nlm.nih.gov/33967047/ and https://clinicaltrials.gov/search?intr=GRF6021
Even with these hurdles, numerous academic and industry-led clinical trials targeting longevity have been initiated. To navigate the long timelines typically required for such research, many trials focus on age-related diseases, such as cardiovascular disease, neurodegeneration, or metabolic disorders, rather than on general life extension. These diseases have more well-defined clinical endpoints, such as the incidence of heart attacks, fibrosis of tissues, or cognitive decline, that can be measured within a reasonable time frame—usually years rather than decades. By targeting diseases closely associated with aging, researchers aim to demonstrate the effectiveness of therapies in extending healthy lifespan, which can then serve as a stepping stone toward broader life extension goals.
Opportunities within Longevity Therapeutics
Despite the significant hurdles, uncertainties and unproven outcomes that characterize the current landscape of longevity therapeutics, our fund believes this area still warrants careful attention. Although many remain skeptical, Luma is an active investor in this space because of the pace at which researchers are unraveling the biological mechanisms of aging—and the potential for these insights to translate into therapies that extend healthspan, creating a breakthrough moment in the near future. Our focus is on identifying companies and technologies that demonstrate not only strong scientific foundations but also clear, realistic pathways from bench to bedside. While we recognize that many questions remain unanswered, we see a measured but meaningful opportunity to invest in advancing this promising frontier of medicine.
One of our earliest investments was in Altos Labs, which we hope will pioneer a new class of drugs for numerous age-related disorders. Their core technology builds on two groundbreaking scientific discoveries. The first is the identification of the Yamanaka factors, which allow any cell type to be reprogrammed into an induced pluripotent stem cell (iPSC) state. This Nobel Prize–winning discovery triggered a wave of stem cell research, providing researchers—including my partner Themasap and me—with the tools to create and study stem cells in the lab. Today, thousands of researchers are capable of generating stem cells and are developing methods to differentiate them into virtually any cell type. We have begun to understand the factors and pathways that enable the conversion of stem cells into every specialized cell in the human body, all in a dish.
Although the discovery of Yamanaka factors and iPSCs revolutionized our understanding of stem cells, this insight, on its own, does not constitute a therapeutic solution. However, it enables the generation of specific cell types ex vivo that could be reintroduced into the body to replace damaged or lost tissues. While many companies are pursuing ex vivo therapies—in which cells are grown outside the body and then injected into diseased or aged tissues to restore function—this approach comes with significant challenges. One major hurdle is scaling up cell production. Producing the billions of specialized cells required to meet clinical and commercial demands is currently very difficult. The process still occurs on a relatively small scale and can suffer from considerable batch-to-batch variability. Additionally, the field has yet to solve the delivery problem. Even if we can generate the necessary cells at scale, we must determine how to precisely deliver them to the affected tissues. These cells often require extremely accurate placement to function properly, making their targeted delivery a complex, and often overlooked, challenge. For instance, some companies are developing ex vivo cell translatplate approaches for Parkinsons’ Disease. This requires developing iPSC-derived dopaminergic neurons to replace those lost in the substantia nigra—a region located deep in the interior of the brain—where delivery of therapeutic cells poses a significant challenge.
The second key discovery for Altos originated in the laboratory of Dr. Juan Carlos Izpisua Belmonte at the Salk Institute for Biological Studies. While Yamanaka factors were originally known for their ability to revert cells all the way back to a stem cell state, the Belmonte lab found a more nuanced approach. Rather than fully resetting a cell’s identity, these factors could be applied in a way that only “partially” reprograms the cell, making it more resistant to stress while preserving its original characteristics. The result is a cell that retains youth’s functional benefits without losing its specialized role in the body. They have seen this work across various tissue types and organs, from skin to kidneys and others.25 Luma remains excited about the progress the Altos has made and believes they will be one of the next generation companies that help redefine longevity.
What does the future of aging look like?
The future of aging therapeutics is poised to revolutionize healthcare, as advances in biotechnology, genomics and drug development converge to target the root causes of aging. Rather than simply managing age-related diseases, emerging therapies aim to intervene at the cellular and molecular levels, promoting longevity and healthier aging. These advancements will take time and will initially focus on areas where we have the deepest scientific knowledge, gradually expanding to other aspects of aging. Progress is already being made in addressing individual age-related diseases, such as fibrosis and cardiometabolic conditions, while researchers work to uncover the science that will unlock solutions for the next wave of age-related conditions. With breakthroughs in areas like senolytics, epigenetic reprogramming and personalized medicine, the next generation of therapeutics could extend healthspan—allowing individuals to live longer, more vibrant lives. As the global population ages, the development of these therapies will play a critical role in reshaping both healthcare and society.
- https://www.embopress.org/doi/full/10.15252/embj.201386907 ↩︎
- https://www.ncbi.nlm.nih.gov/pmc/articles/PMC8903056/ ↩︎
- https://www.ncbi.nlm.nih.gov/books/NBK22252/ ↩︎
- https://www.embopress.org/doi/full/10.1002/emmm.201200245 ↩︎
- https://elifesciences.org/articles/31299 ↩︎
- https://www.nature.com/articles/s41467-019-12664-x ↩︎
- https://www.thelancet.com/article/S2352-3964(15)30081-5/fulltext ↩︎
- https://www.aging-us.com/article/203736/text ↩︎
- https://www.science.org/doi/10.1126/scitranslmed.abd2655 ↩︎
- https://www.tandfonline.com/doi/full/10.1080/00207454.2022.2057849 ↩︎
- https://doi.org/10.1038/s41586-020-03129-z ↩︎
- https://www.pnas.org/doi/full/10.1073/pnas.1516131112 ↩︎
- https://www.nature.com/articles/ncomms3300 ↩︎
- https://www.nature.com/articles/nm.4222 ↩︎
- https://www.nature.com/articles/s41420-020-00365-0 ↩︎
- https://www.science.org/doi/10.1126/science.abe9985 ↩︎
- https://elifesciences.org/articles/60827 ↩︎
- https://elifesciences.org/articles/60827 ↩︎
- https://www.science.org/doi/10.1126/science.abg7292 ↩︎
- https://www.nature.com/articles/s41580-019-0199-y ↩︎
- https://onlinelibrary.wiley.com/doi/10.1111/acel.13028 ↩︎
- https://doi.org/10.1038/s41591-018-0092-9 ↩︎
- https://doi.org/10.1016/j.ebiom.2019.08.069 ↩︎
- https://www.cell.com/cell/fulltext/S0092-8674(06)009767?_returnURL=https%3A%2F%2Flinkinghub.elsevier.com%2Fretrieve%2Fpii%2FS0092867406009767%3Fshowall%3Dtrue ↩︎
- https://www.nature.com/articles/s43587-022-00183-2#auth-Juan_Carlos-Izpisua_Belmonte-Aff2-Aff4 ↩︎